Key Parameters that Define Solar Cell Performance
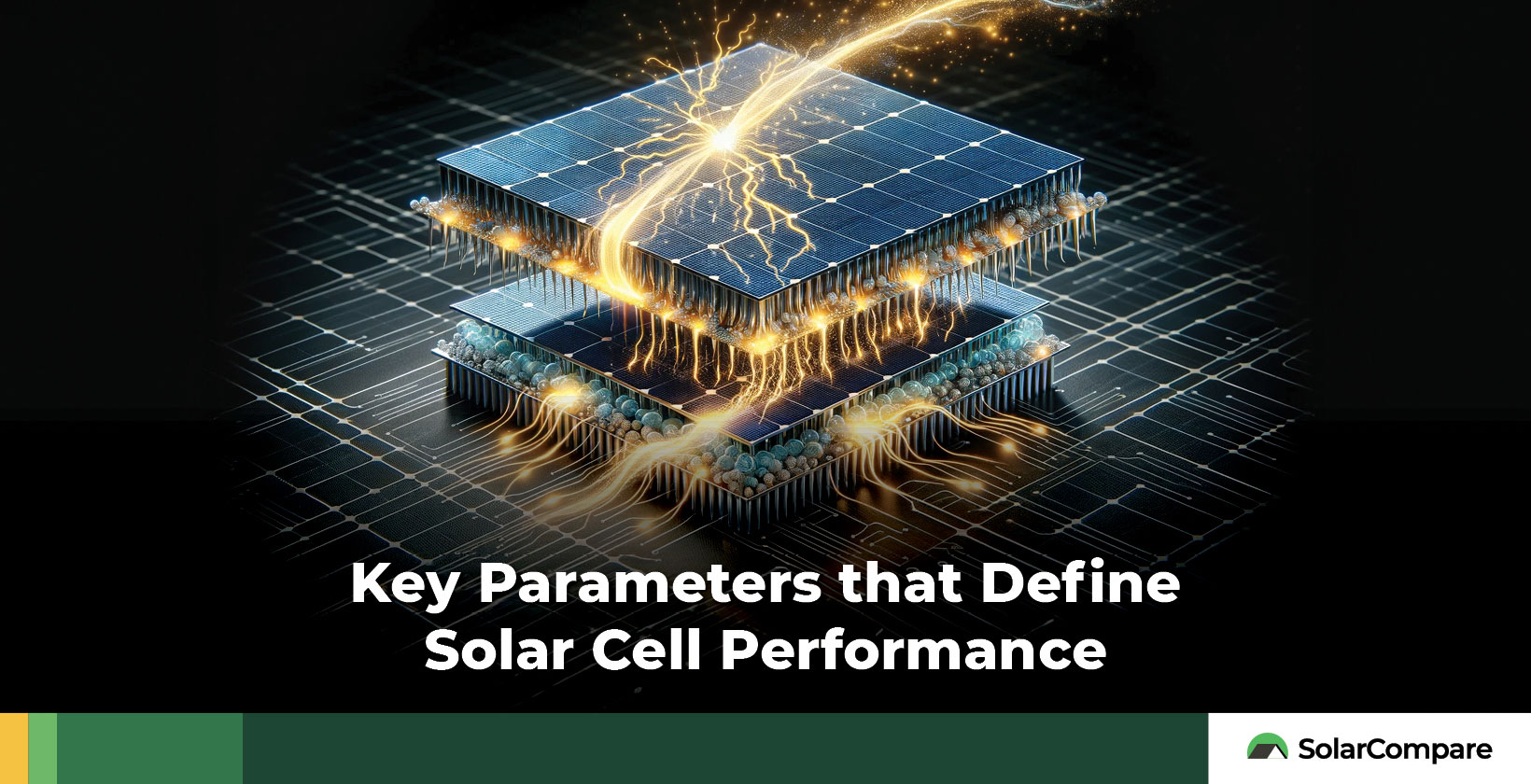
Solar cells, also known as photovoltaic (PV) cells, have several key parameters that are used to characterize their performance. The main parameters that are used to characterize the performance of solar cells are short circuit current, open circuit voltage, maximum power point, current at maximum power point, the voltage at the maximum power point, fill factor, and efficiency.
The short circuit current (ISC) is the maximum current that flows from a solar cell when the voltage across the cell is zero. The open circuit voltage (Voc) is the maximum voltage available from a solar cell when the circuit is open and no external load is connected, allowing the flow of current. The maximum power point (MPP) is the point on the solar cell's current-voltage response curve that generates maximum electrical power output, calculated by multiplying current (I) times voltage (V).
The current at the maximum power point (Impp) is the current (amps) generated by the solar cell at the voltage value corresponding to peak power output on the IV curve. The voltage at maximum power point (VMPP) is the output voltage from the solar cell corresponding to the peak power point along the IV curve. The fill factor (FF) is a measure of the efficiency of the PV module. FF measures solar cell quality by comparing maximum power to the theoretical power available. The efficiency of a solar cell quantifies the percentage of the sun’s energy striking the cell that gets converted into usable electrical energy.
The key parameters defining solar cell and panel performance are important in evaluating device capabilities, guiding technological improvements, enabling appropriate system design, and quantifying manufacturing quality. Solar cells, also called photovoltaic (PV) cells, are devices engineered to convert sunlight directly into electricity using a phenomenon known as the photovoltaic effect. The photovoltaic effect is the process that generates voltage or electric current in a photovoltaic cell when it is exposed to sunlight.
Solar cells have a layered structure consisting of a photosensitive semiconductor material that generates electricity from light. Solar cells work by producing electricity from sunlight through the photovoltaic effect. When sunlight strikes the semiconductor material within the cells, typically silicon, it energizes the electrons. To identify whether a solar cell is working properly, check the indicator light on the solar inverter, inspect the batteries, consider the weather factors, and check the panels for micro-cracks and broken wires.
Solar cell parameters are measured accurately using 6 main methods. These methods are IV curve tracing, quantum efficiency measurement, sun simulators, electroluminescence imaging, temperature characterization, and spectral response measurement.
What are the Parameters of Solar Cells?
Solar cells, also known as photovoltaic (PV) cells, have several key parameters that are used to characterize their performance. The seven main parameters that are used to characterize the performance of solar cells are short circuit current, open circuit voltage, maximum power point, current at maximum power point, the voltage at the maximum power point, fill factor, and efficiency.
More information on each of the 7 key solar cell parameters is below.
1. Short Circuit Current
The short circuit current (ISC) is the maximum current that flows from a solar cell when the voltage across the cell is zero. The ISC of a solar cell measures light-generated current collection with no resistive losses. Under short circuit conditions, the solar cell does not dissipate power since the voltage is zero.
The short circuit current primarily depends on the cell's effective area, spectrum of incoming light, and quantum efficiency. A larger surface area for photon absorption increases short-circuit current. Quantum efficiency determines the fraction of absorbed photons converted to electrical current. Solar cell junction materials and defect densities limit ISC.
Measuring the short-circuit current (ISC) of a solar cell helps determine the maximum current the cell can produce under standard test conditions. A digital multimeter and test leads with crocodile clamps are used to measure the short circuit current. The short-circuit current is the current through the solar cell when the voltage across the solar cell is zero, and it is measured by connecting the positive and negative terminals of the panel to each other through an ammeter in series. This measurement is essential for assessing the overall current generation capacity and the effectiveness of light absorption in the solar cell. A high ISC enables high power density. The ISC decreases slightly with increasing temperature due to changes in charge carrier mobility and recombination effects. Optimized semiconductor doping and transparent conductor selections maximize the value of the short-circuit current.
2. Open Circuit Voltage
The open circuit voltage (Voc) is the maximum voltage available from a solar cell when the circuit is open, and no external load is connected, allowing the flow of current. Voc is measured with a multimeter across the open ends of the wires attached to the panel. Under open circuit conditions, the solar cell does not dissipate power since the current is zero.
The open-circuit voltage (Voc) is the maximum electrical pressure a solar cell creates when it's not connected to anything. The Voc is a measure of the potential energy the solar cell can generate. This voltage increases as the light shining on the solar cell gets brighter but changes with temperature. The open-circuit voltage is lower when the solar cell is very hot, and the voltage is higher when the solar cell is cooler. The open-circuit voltage is an essential parameter for designing solar cells for different uses and understanding how they perform in various conditions.
Measuring open circuit voltage reveals the maximum electric potential solar cells can leverage for doing useful work. Higher Voc enables greater power output and efficiency potential once connected to circuits. VOC response across varying light and temperature depends on subtle bulk and interface energetic properties influenced by materials purity and defect densities.
Assume the open-circuit voltage of a solar cell is optimized by improving the interface passivation. The solar cell is more efficient, making the most of the potential energy it can generate. When connecting multiple cells into modules, open-circuit voltage and current generation must be balanced based on the specific needs of the application they will be used for. This balance will ensure that the electrical system is designed to provide the right amount of power for the devices it will be connected to.
3. Maximum Power Point
The maximum power point (MPP) is the point on the solar cell's current-voltage response curve that generates maximum electrical power output, calculated by multiplying current (I) times voltage (V). The maximum power point (MPP) is expressed as (P=V *I). The MPP represents optimal solar cell operating conditions.
When the voltage across a solar cell increases from zero, the current it produces decreases due to internal resistance, which causes the cell to lose more of the light-generated carriers. As a result, the power initially rises, then reaches a peak, and eventually drops. This behavior is often referred to as the "knee" of the solar cell's performance. To extract the maximum power from the solar cell, match the impedance of the connected load to the cell's impedance at this "knee" point. This typically allows for the extraction of around 80% of the open-circuit voltage (VOC) of the solar cell. The MPP is the sweet spot where the solar cell can deliver the most power and then connect the load in a way that allows it to make the most of this power.
Tracking the maximum power point (MPP) of a solar cell over varying conditions helps reveal its peak performance capabilities. The MPP represents the bias potential at which the solar cell outputs the maximum net power. MPP enables optimally sizing solar systems for target loads. The ratio of actual maximum output power to the product of open circuit voltage and short circuit current defines solar cell fill factor quality.
Maximum power point tracking electronic converters continuously match impedance for peak efficiency delivery to the load. Tight manufacturing consistency control raises normalized MPP. Defining optimal working bias points ensures cells operate at a sweet spot, balancing voltage and current.
4. Current at Maximum Power Point
The current at the maximum power point (Impp) is the current (amps) generated by the solar cell at the voltage value corresponding to peak power output on the IV curve. This point is central to determining overall current operating behavior.
As voltage increases, it reaches a knee where the current begins dropping faster due to building resistance losses. The current at maximum power point actual amperage you want to see when it is connected to the MPPT solar equipment under standard test conditions. Impp marks the onset of that knee, signaling optimal current bias level before substantial power conversion deterioration.
Analyzing the Impp (maximum power point current) of a solar cell or panel helps determine the maximum amount of power it can produce under different conditions. This Impp helps to properly size solar cells and panels for specific loads to avoid over or underutilization. Rating solar modules based on Impp provides a benchmark for their expected real-world energy delivery capacity. Understanding the maximum capability of the solar cell or panel helps to match the power needs without wasting its potential or overloading it.
In practical terms, maximum power point tracking (MPPT) grid inverters are used to capture the highest possible current from solar arrays under changing environmental conditions. The Impp (maximum power point current) quantifies the solar cell's capabilities independently of its connection, similar to the short circuit current minus resistive effects. This means that IMPP helps understand the maximum power a solar cell can provide, regardless of its connection.
Maintaining tight IMPP device tolerances raises normalized peak current production uniformity for manufacturing yield. Optimizing the current at maximum power point balances generation and loss mechanisms to coordinate solar cell and system design.
5. The Voltage at Maximum Power Point
The voltage at maximum power point (VMPP) is the output voltage from the solar cell corresponding to the peak power point along the IV curve. The IV curve is a relationship, typically represented as a chart or graph, between the electric current through a circuit, device, or material and the corresponding voltage, or potential difference, across it.
This voltage represents the optimal DC bias level for extracting maximum electrical work from the cell. As current is drawn from zero short circuit conditions, voltage drops due to internal resistance until reaching a minimum where power peaks. VMPP occurs just past that clear knee sweet spot. Analyzing VMPP provides critical voltage requirements for properly designing solar power systems, setting DC bus voltages, and configuring connections to efficiently utilize cell capabilities. Proper impedance matching ensures load regulation maintains bias near VMPP-enhancing energy delivery.
In practice, maximum power point tracking (MPPT) grid inverters dynamically sample array voltage levels to extract maximum power. VMPP offers an invariant barometer of solar cell bias potentials near ideal standardized test conditions.
Tight voltage at maximum point (VMPP) tolerances for manufacturing consistency improve power normalization and predictable field reliability. Optimizing the intricate balance between voltage, current, and resistance at the critical maximum power point unlocks performance gains.
6. The Fill Factor
The fill factor (FF) measures the efficiency of the PV module. It measures solar cell quality by comparing maximum power to the theoretical power available. Fill Factor is the ratio of peak electrical power produced divided by the product of open circuit voltage and short circuit current.
The fill factor (FF) of a solar cell is calculated by dividing the peak electrical power produced by the product of the open circuit voltage and short circuit current. The FF ranges from 0 to 1, with 1 representing a perfect solar cell. Typical values range from 0.70 to 0.85 for commercial silicon cells. A higher fill factor signals that the cell converts absorbed sunlight into usable electric energy more efficiently.
A simple way to understand the fill factor of a solar cell is how rectangular the solar cell's current-voltage curve appears. A perfect square would have a maximum area with high voltage and current, meaning an excellent fill factor near 1. Curves that sag down early have smaller areas and power output, resulting in a poorer fill factor.
Maximizing fill factor enables solar cells to deliver capabilities closer to theoretical generation in real operating conditions. Key methods to raise the FF of a solar cell include improving material quality to reduce losses and optimizing grid contact design to lower resistance. Maintaining tight tolerance consistency also aids manufacturing yield.
Overall, the fill factor quantifies inherent solar cell operating behavior integrity independent of exact test conditions influencing measured voltages and currents. It is useful for quality benchmarking and it is a key factor in determining the maximum power from a solar cell.
7. Efficiency
The efficiency of a solar cell quantifies the percentage of the sun’s energy striking the cell that gets converted into usable electrical energy. Solar cell efficiency is the ratio of peak electrical power output divided by the incident light irradiance power.
The efficiency of a solar cell depends on individual solar cell design properties and materials that influence how many photons get absorbed and how much of the absorbed light gets converted to electricity. The efficiency of solar cells generally varies from 6-44%. State-of-the-art silicon solar cells achieve around 20% efficiency.
Higher solar cell efficiency enables the use of fewer panel areas to capture the desired amount of power. Improving efficiency directly makes solar electricity cheaper per watt by reducing the required number of cells. Almost all solar cost reduction stems from increased cumulative production efficiency gains over decades.
Raising efficiency requires optimizing light absorption across solar spectral irradiance while minimizing process losses like resistance and carrier recombination. Advanced materials like multi-junction III-V cells reach over 40% efficiency by targeting different energy photons.
Overall, solar cell efficiency measures how effectively a solar cell converts sunlight into usable electrical energy. It is the ratio of the energy output from the solar cell to the input energy from the sun. The ultimate efficiency potential for typical single-junction silicon cells is around 30% due to the laws of physics and how solar cells absorb power. The efficiency of a solar panel is always lower than that of its component cells due to the frame, reflective metal contacts, and gaps between the cells. For silicon solar cells, the most common type, the theoretical limit is about 32%, but mass-market solar cells typically achieve a maximum efficiency of up to 25%.
What is the importance of solar panel parameters?
The parameters defining solar cell and panel performance are important in evaluating device capabilities, guiding technological improvements, enabling appropriate system design, and quantifying manufacturing quality.
Solar cell parameters like efficiency, voltage, current, and fill factor reveal how effectively the fundamental light-to-electricity conversion process occurs. Economic metrics reflect production cost reductions essential for solar adoption. Analyzing parameters over environmental stress exposure accelerates reliability assessments. Comparing parameters informs optimization balancing interconnected loss mechanisms.
Standardized testing methodology allows performance benchmarking progress historically across research labs and commercial products. Accurate solar panel datasheets with electrical specifications, dimensions, and mechanical attributes support systems design for properly tailored photovoltaic integration. Overall, tracking comprehensive operational parameters of solar cells provides critical insight into solar product design, quality, and field value essential for continuous technological improvement.
What are Solar Cells?
Solar cells, also called photovoltaic (PV) cells, are devices engineered to convert sunlight directly into electricity using a phenomenon known as the photovoltaic effect. The photovoltaic effect is the process that generates voltage or electric current in a solar cell when it is exposed to sunlight.
When photons from sunlight strike the solar cell, they transfer their energy to the PV material, freeing electrons from the atom of the material. This generates electron-hole pairs that get swept across an internal electrical field at a p-n junction in the cell, producing electric current and voltage. Metallic contacts guide this photo-generated electrical output to external wiring for power utilization.
Solar cells are the basic building blocks of solar panels. Solar cells are responsible for converting sunlight into electricity. They work by using a process called the photovoltaic effect, which allows them to harvest energy from sunlight. When connected together, these solar cells form modules, panels, and arrays, which can be scaled up to meet the specific electricity needs of different applications. While there are various types of solar cell technologies, they all rely on semiconductors with unique light-sensitive properties, p-n junctions for controlling the flow of electrical current, anti-reflection coatings to minimize light loss, and contact layers to efficiently extract electricity from solar photons.
What is the Structure of Solar Cells?
Solar cells have a layered structure consisting of a photosensitive semiconductor material that generates electricity from light. The semiconductor material is encased within electrical contacts. Electrical contacts are electrically conductive material, typically metal, in electrical switches, relays, connectors, and circuit breakers. These semiconductor materials are usually silicon cells that absorb sunlight and generate an electrical current.
Typically, the silicon cells are composed of two primary layers - a negative electron-rich n-type silicon layer on top and a positive hole-rich p-type silicon layer on the bottom. An electrical p-n junction forms between the layers. A p-n junction is a boundary or interface between two types of semiconductor materials, p-type and n-type, inside a single crystal of semiconductor. The “p” (positive) side contains an excess of holes, while the “n” (negative) side contains an excess of electrons. On top of the cell, an anti-reflective coating maximizes light absorption and minimizes sunlight glare reflection. Metal contact gridlines across the top collect photogenerated electrons, while rear contacts collect holes for current flow.
What is the principle of a Solar Cell?
The principle of a solar cell is based on the photovoltaic effect, which is the process by which sunlight is converted into electricity. Solar cells, also known as photovoltaic cells, are semiconductor devices designed to harness this effect. When sunlight hits the solar cell, the energy from the light excites electrons in the semiconductor. This creates pairs of electrons and ‘holes,’ with the ‘holes’ effectively acting as positive charge carriers. An internal electric field within the solar cell separates these electron-hole pairs, causing the electrons to move toward one side (creating a negative charge) and the holes towards the other (creating a positive charge). When the circuit connected to the solar cell is closed, the electrons flow through the external circuit to fill the holes on the other side, creating an electric current. A solar cell is the fundamental component of a photovoltaic module. A photovoltaic module is an array of solar cells that, in combination, generate enough electricity to power homes and businesses.
What are the Types of Solar Cells:
The four main types of solar cells are monocrystalline, polycrystalline, thin-film cells, and PERC cells. The type of solar cell typically corresponds to the main solar panel types, influencing key factors such as efficiency, output, cost, and appearance. More information on the 4 major solar cell types is below.
- Monocrystalline Solar Cells: Monocrystalline solar cells, often referred to as "mono cells," are crafted from single-crystal silicon, making them highly efficient. They are recognizable by their uniform, dark appearance, and rounded edges. Monocrystalline cells offer superior energy output due to the unhindered movement of electrons within the single silicon crystal layer. These cells are known for their longevity, lasting up to 40 years with proper maintenance. However, they tend to be more expensive upfront compared to other types. Leading manufacturers like Canadian Solar and Astronergy produce monocrystalline cells with efficiency rates of up to 23%.
- Polycrystalline Solar Cells: Polycrystalline, or multicrystalline, solar cells are manufactured by melting together multiple silicon crystals. They are less efficient than monocrystalline cells but are more cost-effective. Polycrystalline cells have a characteristic blue hue and offer moderate efficiency (13-16%). They are commonly used in residential and commercial applications due to their affordability and versatility.
- Thin-Film Solar Cells: Thin-film solar cells are produced by depositing photovoltaic elements over a surface, such as glass, plastic, or metal. They are flexible and can be applied in various settings, including solar farms, vehicles, and portable devices. The subtypes of thin-film solar cells include cadmium telluride (CdTe) cells, copper indium gallium selenide (CIGS) cells, and amorphous silicon (a-Si) cells. While they have lower efficiency (around 7-22%) compared to crystalline cells, they offer advantages such as lower manufacturing costs and improved heat tolerance.
- Passivated Emitter and Rear Cell (PERC) Solar Cells: PERC solar cells are a modified version of traditional silicon cells, featuring an additional layer on the back panel. This layer allows PERC cells to produce more energy than conventional silicon cells by reducing efficiency losses. PERC technology has led to higher conversion efficiency (up to 1-2% increase) and an extended lifespan due to lower heat generation. However, PERC cells may be susceptible to Light-Induced Degradation (LID) and Potential Induced Degradation (PID), which can impact their long-term performance.
Beyond the four solar cell types commonly found in solar panels, there are a number of other “third-generation” solar cells that have been developed in recent decades. These include Dye-Sensitized Solar Cells (DSSC), Quantum dot-sensitized Solar Cells (QDSSCs) and Pervoskite Cells.
How Do Solar Cells Work?
Solar cells work by producing electricity from sunlight through the photovoltaic effect. When sunlight strikes the semiconductor material within the cells, typically silicon, it energizes the electrons. These excited electrons are then collected and flow through an external circuit, creating a Direct Current (DC) electrical flow. This process of cells absorbing sunlight and producing an electrical current is the fundamental principle of how a solar panel works.
First, photons from incoming sunlight strike the solar cell. If the photons have enough energy, they can transfer that energy to electrons floating inside the solar cell's semiconductor material, like silicon. That energy transfer frees the electrons from their atoms, creating electron-hole pairs. The electrons move freely, but the holes act like positive charges fixed in place.
Then, the solar cell has an electric field across its positive and negative sides that forms a pathway pulling the free electrons away from their holes. This field comes from joining two types of silicon together to make a p-n junction diode.
Next, metal contacts on the cell collect all the electrons and holes and transmit them into external wires, where they become electric currents and voltage power a load.
Finally, the process runs continuously as long as sunlight shines on the solar cell, constantly generating more electron-hole pairs and current flow without being used up.
How To Identify If a Solar Cell is Working Properly?
The primary indicator to identify whether a solar cell is working properly is to check if it is producing its expected electrical output under standard test conditions. A monocrystalline silicon solar cell at 25°C temperature receiving 1 kW/m2 simulated solar illumination should generate between 0.5-0.6 volts of open circuit voltage and over 30 milliamps of short circuit current density. Measuring electrical parameters such as operating voltage, operating current, fill factor, and conversion efficiency quantifies solar cell performance versus specifications. Additional checks under varying controlled lighting levels, temperatures, and spectra reveal detailed signatures of healthy behavior.
Beyond electrical tests, imaging inspections determine cracks, connectivity flaws, or mechanical defects. By comparing key metrics such as maximum power point current-voltage values against predetermined baselines at standard reporting conditions, solar cells are easily characterized to confirm proper operation or detect substandard performance requiring replacement. When electrical response, visual examination, and production records affirm that the solar cells reach the standard quality expectations, then the solar cells can be verified as functional.
To identify if a solar cell is working properly, check the indicator light on the solar inverter, inspect the batteries, consider the weather factors, and check the panels for micro-cracks and broken wires.
How long do Solar Cells last?
Most solar cells and panels are designed to last around 25-30 years or more. Manufacturers typically guarantee at least 80% power output for 20-25 years.
The solid and healthy construction of solar cells provides weather and corrosion resistance, allowing long-term exposure. Higher temperatures, moisture ingress, mechanical stresses, and ultraviolet irradiation accelerate the long-term degradation of solar cells. Lab testing simulating 30 years of field conditions predicts a loss of 1-3% annual solar cell efficiency. Strategies like glass superstrate for stiffness, robust edge seals, protective polymer coatings, and quality contact metallization enhance module life expectancy.
Careful transportation, installation, maintenance, and operation also prevent premature failure. Using conservatively rated components ensures the power thresholds of solar cells stay above warrantied ratings. While catastrophic events can randomly impact the probability of survival, statistical models guide retirement-for-age based on the asymptotic decay of large populations. With sound engineering and manufacturing, crystalline silicon panels may operate for 50 years in temperate climates, according to researcher John Fitzgerald Weaver at the PV Magazine USA. Overall, proven robust integrated mechanical design enables multi-decade functional reliability.
Can Solar Cell Parameters Be Measured Accurately?
Yes. Solar cell parameters can be measured accurately. Solar cell parameters are the electrical characteristics of a solar cell, such as Open-circuit voltage (Voc), Short-circuit current (Isc), Maximum power point (Vmp), The Voltage at Maximum Power Point, Fill Factor, Efficiency, Current at Maximum Power Point (Imp) are measured with high accuracy using specialized equipment like solar cell I-V testers. These testers usually operate under Standard Test Conditions (STC), meaning specific irradiance, spectrum, and cell temperature. This ensures consistent and comparable measurements.
Measuring these solar cell parameters accurately is essential for characterizing, optimizing, and evaluating the performance of solar cells and modules. However, there are many factors that can affect the accuracy of solar cell parameter measurements. These factors are the type and quality of the measurement, environmental conditions, capacitance effects of high-efficiency solar cells, and the series resistance of the solar cell.
The type and quality of the measurement equipment and instruments are the light source, the reference cell, the load, the voltmeter, the ammeter, and the data acquisition system. The environmental conditions are the temperature, the humidity, the irradiance, the spectrum, and the angle of incidence of the light. The capacitance effects of high-efficiency solar cells and modules can cause transient responses and distortions in the current-voltage curves. The series resistance of the solar cell, which can reduce the fill factor and the output power of the cell.
What Methods Are Used to Accurately Measure Solar Cell Parameters?
Solar cell parameters are measured accurately using 6 main methods. These methods are IV curve tracing, quantum efficiency measurement, sun simulators, electroluminescence imaging, temperature characterization, and spectral response measurement.
More information on each of the 6 main methods of measuring solar cell parameters accurately is below.
1. IV Curve Tracing
IV Curve Tracing is a performance verification method used in photovoltaic (PV) systems. An I-V curve is a graphical representation of measured current (I) and power as a function of voltage (V). The IV Curve Tracing method is integral to accurately measuring PV module performance and diagnosing degradation in power output.
IV Curve Tracing allows for the measurement of current and voltage when the module is under load, providing non-invasive diagnostics for PV module performance. By measuring the I-V curve of your solar panels, you accurately predict how much energy your system is expected to generate, which is crucial for planning and optimizing your energy usage. When you analyze the measured I-V curve against the expected curve, you identify internal and external faults such as a damaged solar cell, shaded areas, increased temperature strains, or defective bypass diodes.
The accuracy of the IV Curve Tracing method is high as it allows for both current and voltage measurement when the module is under load. The accuracy of IV Curve Tracing is influenced by environmental conditions during data capture. IV Curve Tracing remains a reliable method to accurately measure the performance of photovoltaic (PV) systems.
2. Quantum Efficiency Measurement
Quantum Efficiency (QE) measures the effectiveness of a photovoltaic (PV) device, such as a solar cell, to convert incident photons into electrons. A sensor with a QE of 100% and exposed to 100 photons would produce 100 electrons of signal.
Quantum Efficiency (QE) measurement is one of the most significant characterization tools for solar cells, allowing for quantifying the efficiency of the conversion of light to electrons as a function of the wavelength of the impinging light. The QE measurement indicates how well the solar cell converts sunlight to electricity. A solar cell is expected to have an optimal spectral response at the point at which the spectral component of sunlight is abundant. The QE has a value of 100% if all the photons of a specific wavelength are absorbed and the resulting carriers are collected. The QE for most solar cells is reduced because of the effects of recombination, where charge carriers cannot move into an external circuit.
The accuracy of QE measurement is optimized by configuring gain and range to detect the low-level modulated current from the PV cell3. The repeatability for stable p-n junction solar cells is better than ±0.3 % in the 400 nm to 1000 nm range and better than ±0.6 % in the 300 nm to 400 nm and 1000 nm to 1100 nm ranges.
3. Sun Simulators
Sun Simulator, also known as an artificial sun or sunlight simulator, provides illumination that approximates natural sunlight. The purpose of the Sun Simulator is to provide a controllable indoor test facility under laboratory conditions. Sun simulator is used to test any photosensitive processes or materials, including solar cells. Photosensitivity is when a surface becomes very sensitive to sunlight or other forms of ultraviolet light.
Sun Simulators work by measuring the efficiency of solar cells and modules. A solar source that effectively mimics the spectrum of the sun is used to characterize how solar cells will perform in the real world. Sun Simulators need specific lamps to simulate the solar spectrum and a specific filter to simulate atmosphere absorbance. These standards specify the following dimensions of control for light from a solar simulator: spectral content (quantified as spectral match), spatial uniformity, and temporal stability.
The accuracy of Sun Simulators is high as they provide a controllable indoor test facility under laboratory conditions. The standards specifying performance requirements of solar simulators used in photovoltaic testing are IEC 60904-9, ASTM E927-19, and JIS C 89121. These standards specify the dimensions of control for light from a solar simulator: spectral content (quantified as spectral match), spatial uniformity, and temporal stability.
4. Electroluminescence Imaging
Electroluminescence (EL) Imaging is a non-destructive testing method used in photovoltaics. EL involves the emission of light from a material when an electric current is passed through it or when it is subjected to a strong electric field. This technique detects defects and inefficiencies in solar cells and modules.
Electroluminescence Imaging provides a wealth of data about the area-related uniformity of solar cells and modules. It is sensitive to the effects of shunt and series resistance and recombination parameters. The light output increases with the local voltage, so regions with poor contact appear dark. EL is used to identify areas of a solar cell that are underperforming or malfunctioning.
Electroluminescence Imaging is a highly accurate method for characterizing solar cells. EL detects micro-cracks and printing problems that are not detectable with visual inspection. The process speed of each picture is 8.07 ms per picture, and the accuracy is 93.02%.
5. Temperature Characterization
Temperature characterization involves measuring how the performance of a device or system, such as a solar cell, changes with temperature. Temperature characterization helps understand and optimize the performance of devices that operate under varying temperature conditions.
Temperature characterization provides valuable insights into the performance of solar cells. Temperature characterization identifies how the efficiency of a solar cell changes with temperature. Solar cells heat up under sunlight, and their efficiency decreases as the temperature increases. By characterizing these changes, you optimize the design and operation of solar cells for different environmental conditions.
The accuracy of temperature characterization methods can be high, but it depends on the specific techniques and equipment used. Thermopiles, which are arrays of thermocouples, measure the temperature difference between the irradiated side and the side that is in the dark. Thermopile is an electronic device that converts thermal energy into electrical energy. This measurement between the irradiated and dark sides is useful for characterizing the solar resource for solar thermal collectors or comparing the performance of solar thermal and solar photovoltaic systems.
6. Spectral Response Measurement
Spectral Response Measurement is used to characterize the efficiency of a solar cell at different wavelengths of light. The spectral response is conceptually similar to quantum efficiency. The quantum efficiency gives the number of electrons output by the solar cell compared to the number of photons incident on the device. The spectral response is the ratio of the current generated by the solar cell to the power incident on the solar cell.
Spectral response measurement is essential since it is the spectral response that is measured from a solar cell and used to calculate the quantum efficiency of the cell. Quantum efficiency is determined from the spectral response by replacing the power of the light at a particular wavelength with the photon flux for that wavelength. This method is integral to accurately measuring the performance of the photovoltaic (PV) module and diagnosing degradation in power output.
The accuracy of Spectral Response Measurement methods can be high, but it depends on the specific techniques and equipment used.
Are Solar Cells the Main Conductors of Electricity Generation?
No, solar cells are not the main conductors of electricity generation. Solar cells, also known as photovoltaic (PV) cells, are devices that convert sunlight directly into electricity. They do this through the photovoltaic effect, where they absorb photons (light particles) and generate a flow of electricity. Photovoltaic (PV) cells are not conductors in the traditional sense.
A conductor is a material that allows electric charge to move freely. This movement of electric charge is what forms an electric current. Metals like copper and aluminum are common conductors used in electrical systems. Solar cells contain a material called a semiconductor, usually silicon. Semiconductors are unique because they can conduct electricity under certain conditions - in this case, when sunlight provides the energy. While solar cells play a crucial role in generating electricity from sunlight, they do so as semiconductors, not conductors.
Are Solar Cells Ineffective After Their Lifespan?
Yes, solar cells do become less effective after their lifespan. Solar panels, made up of solar cells, typically have a 25-30-year lifespan. Over time, they naturally degrade and produce less energy, a process known as the degradation rate. Solar cell degradation is a key factor in determining how long solar panels last. Solar degradation means that solar panels tend to lose their efficiency over time, typically quite slowly at a rate of 0.8% per year. Most solar panels do not stop producing electricity completely, even after their rated lifespan. Solar panels continue to generate power but at a reduced rate. While solar panels become less effective, they are not entirely ineffective.